Currently, despite decades of trial and error, peripheral nerve injury is an impenetrable clinical dilemma. Any proven effective pharmacologic agent leads to a decisive leap forward to the clinical management of neuropathies. This study investigated the effects of tacrolimus and erythropoietin on sciatic nerve regeneration. Twenty-three mice were randomly assigned to tacrolimus, erythropoietin, tacrolimus + erythropoietin, control, and sham groups following sciatic nerve crush via hemostatic forceps. Medications were administered for 28 consecutive days. The sham group received neither crush injury nor medication. Histopathologic, immunohistochemical, and walking track analyses were performed. In the erythropoietin group, axonal swelling was significantly reduced and the average axonal number significantly recovered up to 75% of normal nerve compared to other groups. Marked immunoreactivity to GFAP and S-100 protein was present in the tacrolimus group. Nevertheless, at least moderate GFAP and S-100 expressions were observed in all of the groups. Functional recovery was superior in the tacrolimus group after 14 days, although a complete return to near-normal function was achieved in all groups after 28 days, regardless of the medication used. Our data supported the neurotrophic effects of tacrolimus and erythropoietin; however, not enough data was gathered to confirm their synergistic effects. Whether these results are extensible to clinical scenarios requires further detailed investigations.
Nowadays, peripheral nerve injury is a substantial source of morbidity, disability, and economic burdens (
1, 2). The extensive network of nerves throughout the body makes them prone to trauma (
3). One to three percent of the patients are the victims of traumatic incidents, most commonly of vehicle accidents (
1, 4, 5). Other traumatic etiologies including sharp lacerations and fractures lead to penetrating, stretching, and compressive nerve injuries (
2, 6). Regardless of the injury cause, some generalities exist about nerve injuries’ nature and prognosis (
7). Contrary to the central nervous system (CNS), peripheral nerves intrinsically regenerate but their spontaneous restoration capacity is often limited (
1, 4, 6). The recovery depends on multiple patient-related or injury-related variables (
7, 8, 9). The outcomes are better in single-function nerves, early repair, younger patients, milder injuries, and when the repair site is in close proximity to the target muscle (
7, 8, 10). Despite the deployment of accessible surgery-based or supportive treatments, only about 50% of the patients achieve admissible functional retrieval (
6, 8, 10). Even patients treated adequately, often face poor clinical outcomes (
5, 10) including temporary/long-term loss of function and intractable neuropathic pain (
2, 10, 11).
Myriads of putative beneficial pharmacologic agents have been investigated as candidates for reducing neuronal death and promoting axonal outgrowth (
1, 2, 9). Due to the high prevalence of sciatic crush injury in normal life (
12), several studies have explored different substances on the sciatic crush model, possibly aiming to generalize their outcomes to clinical practice (
13, 14, 15). Nevertheless, true and potent therapeutic agents capable of being translated into human medicine are still lacking (
11, 13).
Tacrolimus (FK-506), a calcineurin inhibitor from the macrocyclic lactone group has become the cornerstone of immunosuppressive therapy after organ transplantation due to its great potency and few side effects (
16, 17, 18). However, previous studies suggest that sub-immunosuppressive doses of tacrolimus exhibit substantial neuroregenerative properties (
9, 17). It has been shown that tacrolimus increases neurite elongation, induces cell proliferation, and accelerates the rate of axonal regeneration (
20, 21, 24). Nevertheless, the exact mechanism by which FK-506 exerts its neuroregenerative effects, the most effective dose and duration of treatment remain unclear (
17, 20, 21).
Erythropoietin is an FDA-approved drug used for treating anemia induced by chronic kidney failure and chemotherapy since 1990 (
7). It was originally described as a hematopoietic renal cytokine (
21, 22). However, its recently established neuro-regenerative effects, limited side effects, and safety of therapeutic doses have made erythropoietin an appealing candidate for adjunct treatment in nerve injuries (
7, 21, 22). Erythropoietin stimulates axonal regrowth and encourages angiogenesis (
7, 21). It promotes cell survival by inhibiting apoptosis, alleviates inflammation, protects against oxidative damage, and directly stimulates other neuroprotective molecules such as BDNF (
7, 22, 23). These beneficial effects are particularly significant in concurrent nerve damage and anemia as in patients with multiple traumas and patients undergoing reconstructive joint surgery (
7).
Among experimental models, the rodent sciatic crush model has provided new insights due to its high regenerative capacity, rapid reliable recovery in a predictable period, similar repair process to humans, and simple surgical approach. Additionally, it allows the evaluation of functional recovery, thus being applicable in clinical practice (
12, 24, 25). Although axonal regrowth may be slow, functional recovery following sciatic crush is complete (
4, 10). Recent reports indicated that several compounds could accelerate functional recovery, however, the most effective one has not been discovered yet (
4, 9). The sciatic functional index (SFI) is a popular measure for evaluating functional recovery following nerve injury. Despite its inherent shortcomings, it has been considered the gold standard because of its reliability, repeatability, non-invasiveness, cost-effectiveness, and correlation to indirect methods of measuring functional recovery (
12, 15, 25).
Studies in the field of nerve regeneration have addressed both tacrolimus (
16, 17) and erythropoietin (
7, 23), However, no consistent and practical information can guide their clinical use for managing peripheral nerve injury heretofore. The diversity of animal models and experimental methods is the potential cause of the aforementioned statement. To the best of our knowledge according to reviewed resources, the effect of simultaneous administration of tacrolimus and erythropoietin on the sciatic nerve has not been studied yet. Therefore, this study aimed to explore the independent and possible synergistic effects of these drugs on functional recovery and regeneration of the sciatic nerve. We hypothesized that a combination of both drugs may act synergistically in accelerating sciatic nerve regeneration.
MATERIAL AND METHODSExperimental design and operative procedure
Animal experiments were approved by the Research Ethics Committees of Islamic Azad University-Science and Research Branch on 16/6/2021 (approval ID: IR.IAU.SRB. REC.1400.023). A total of 23 adult male Swiss mice weighing approximately 30 gr were used in the study. Animals were kept in soft wood-chiplined plastic cages one week before the procedure for adaptation purposes. During the experiment, animals were exposed to a 12-hour light-dark cycle and standard rodent chow and water ad libitum were provided. Following animal randomization into groups of five for the experimental groups and three for the sham group, a color code was applied to each animal’s tail to allow blinded identification. The surgery was performed under aseptic conditions using an operating microscope ((Topcan, OMS 90, Tokyo, Japan). The mice were anesthetized with intramuscular injection of 30 mg/kg ketamine hydrochloride (Alfasan International BV, Woerden, The Netherlands) and 0.2 mg/kg medetomidine (Spain SYVA s.a.u, Leon, Spain) to induce sciatic nerve crush according to previously reported protocol with slight modifications (
23, 26). Briefly, the left lateral thigh was prepared aseptically; the sciatic nerve was exposed through a 1 cm gluteal muscle splitting incision and crushed for 10 seconds using fine straight mosquito hemostatic forceps (Schreiber, Fridingen, Germany) 5 mm proximal to the sciatic nerve trifurcation. Complete crush was confirmed by the presence of a translucent band across the nerve. The area was marked with a single 5-0 nylon suture. The muscle layer and skin were approximated routinely. Animals were treated with either 5 mg/kg oral tacrolimus (NanoAlvand Co., Alborz, Iran), 5000 U/kg, subcutaneous erythropoietin (Pooyesh Darou Biopharmaceutical Co., Tehran, Iran), or both single time a day for 28 consecutive days. These dosages were the most effective ones reported in previous studies (
7, 16, 21). The control group received an equivalent volume of subcutaneous 0.9% saline (Shahid Ghazi pharmaceutical CO., East Azerbaijan, Iran) as a standardization measure for the procedure. Sham-operation mice were submitted to sciatic nerve exposure without crushing and received no medication postoperatively.
Tacrolimus preparation and administrationTacrolimus was prepared by the method previously described by Que et al. (
27). Briefly, capsules containing 1 mg tacrolimus were used. Capsule dressing was removed, the powder was dissolved in saline and prepared into a homogeneous suspension. Mice were intragastrically injected with the suspension.
Walking track analysisSFI was measured preoperatively in order to obtain a baseline analysis and on days 1, 14, and 28 following the surgery. Mice’s hind legs were painted with blue ink and the animals were placed in a 49x15x8 cm apparatus darkened at one end. The bottom of the apparatus was covered with appropriately trimmed white A4 paper. The SFI was calculated by the Inserra et al. formula (
28). Theoretically, an index value of 0 represents normal functioning whereas -100 indicates total loss of function.
Histopathologic and immunohistochemistry evaluationFour weeks after the surgery, the animals were sacrificed with an overdose of pentobarbital (300 mg/kg). The sciatic nerve was exposed, dissected from the surrounding tissues, and approximately 5 mm sections of the sciatic nerve containing areas proximal and distal to the crush site were harvested carefully. Samples were fixed in 10% neutral buffered formalin solution for 24 hours, dehydrated through an ethanol series (70-100%), embedded in paraffin, and 5 μm thick longitudinal serial sections were obtained and stained with hematoxylin and eosin to uncover histopathological elements of tissue. In addition, cross-sections of the nerve were stained with toluidine blue to evaluate the nerve architecture and quality of regenerated fibers and to discover elements of tissue inflammation. Nerve regeneration was assessed from the point of defined indices.
The expression of the glial fibrillary acidic protein (GFAP), a useful marker of glial cells in both CNS and PNS, was investigated as a hallmark of Schwann cells and astrocytes activation in the process of nerve regeneration. In addition, anti-S-100 was used as a marker for the myelin sheath that is expressed in peripheral neurons and Schwann cells. Prior to immunohistochemistry, nerve sections were dewaxed, conventionally rehydrated, and then washed with phosphate-buffered saline (Ph 7.4). For antigen retrieval, samples were heated to 95 °C in Tris-EDTA buffer for 20 minutes. For S-100 staining, anti-s-100 (1:100, GenomeME, Canada) and for GFAP staining, GFAP (1:200, GenomeME, Canada) were used. Samples were incubated with 0.6% hydrogen peroxide for 30 minutes to inactivate endogenous peroxidase and then rinsed with phosphate-buffered saline. To block non-specific immunoreactions the sections were incubated with 5% normal goat serum (SinaBiotech, Tehran, Iran). For S-100 staining, sections were incubated in S-100 protein antibody solution for 1 hour at room temperature. They were washed 3 times with phosphate-buffered saline and incubated in biotinylated anti-mouse rabbit immunoglobulin G solution for 1 hour. Horseradish peroxidase–labeled secondary antibody was applied for 1 hour.
For GFAP staining, the specimens were incubated with primary monoclonal antibody for 24 hours at 4 °C and then washed with phosphatebuffered saline solution. Samples were then incubated with Rhodamine conjugate secondary antibody for 2 hours at 37 °C.
Afterward, all sections were incubated with 3,30-diaminobenzidine tetrahydrochloride chromogene substrate (DAB) solution for 10 minutes. Immunohistochemical-stained slides were examined under light microscopy. Staining intensity was rated as negative (-), weakly positive (+), moderately positive (++), and markedly positive (+++) based on an approach described previously (
24).
Macroscopic evaluation
A gross macroscopic assessment was performed on day 28. The presence of selfmutilation, local infection, and inflammation were inspected. Furthermore, muscle adhesion, tissue derangement, adherence of the nerve to the surrounding muscles, muscle fascia closure as well as the skin closure were evaluated by qualitative scoring as previously described (
29) with the modifications indicated below. Concerning muscle adhesion, negative (-) reflected no muscle adhesion, whereas weak (+), moderate (++), and marked (+++) indicated minimal, moderate and severe adhesion, respectively. Tissue derangement was compared between groups so that negative (-) reflected no tissue derangement, weak (+) reflected the lowest degree of derangement, and marked (+++) reflected the maximum degree. Regarding nerve adherence to the surrounding muscles, negative (-) indicated that the nerve was either free or required minimal blunt dissection to separate. Moreover, weak (+), moderate (++), and marked (+++) reflected that mild blunt dissection, moderate blunt dissection, and sharp dissection with scissors was required for separation, respectively. Regarding muscle fascia and skin closure, (+) reflected complete closure and (-) reflected failure of the wound to close.
Statistical analysis
Statistical analyses were done by SPSS software version 26.0. The descriptive statistics were described by mean ± SEM. One-Way ANOVA and LSD post hoc were analyzed each time. The Repeated Measures with a 95% confidence interval were carried out by the general linear model (GLM) procedure for times trends measures. A p-value less than 0.05 was considered statistically significant.
RESULTSFunctional evaluationPreoperatively, the SFI values were measured around -7.5, which is considered near normal function. After surgery, the SFI decreased dramatically to values around -100, indicating a complete loss of function. According to the repeated measures with a 95% confidence interval, SFI values trends of all of the groups were significantly different in measured times (p<0.001) except for the sham group (p=0.068). In Addition, SFI values on day 1 following crush injury were significantly different relative to the baseline values (day zero) in all of the groups, again, except the sham group (p=0.600), characterizing the effectiveness of nerve crushing. SFI values for the sham group were near zero at all time points. There was no significant difference between the sham group SFI values on days 14 and 28 (p=0.077) verifying the validity of the study. Based on repeated measures with a 95% confidence interval, SFI values on day 1 were significantly different from the values on day 14 (p=0.011) and day 28 (p<0.01) in the control group. In addition, the comparison between SFI values on days 14 and 28 with the values on day 1 revealed a statistically significant difference between the tacrolimus, erythropoietin, and tacrolimus + erythropoietin groups (p≤0.001) (
Table 1).
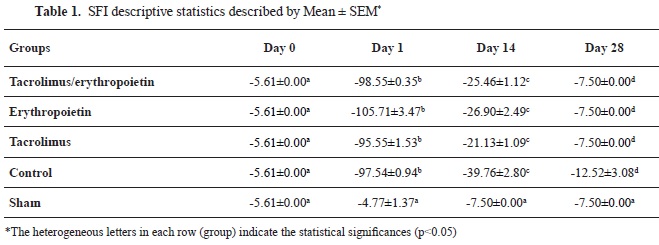
According to the One-way ANOVA test, a statistically significant difference was noted among the groups on day 14 (p<0.001). At this -26.90±2.49, -25.46±1.12, and -39.76±2.80 in the tacrolimus, erythropoietin, tacrolimus + erythropoietin, and control groups, respectively (
Table 1). Superior recovery of the SFI in thetime point, SFI values were returned to -21.13±1.09, tacrolimus group may represent the earlier onset of functional recovery in this group. Furthermore, intragroup assessment using LSD Post Hoc analysis demonstrated a statistically significant difference between tacrolimus and erythropoietin groups (p=0.047). Additionally, the functional return was slightly better in animals treated with both tacrolimus and erythropoietin, although significance wasn't observed between tacrolimus + erythropoietin and either of the tacrolimus and erythropoietin groups at this time point (p=0.127). Moreover, there was a significant difference between the control and experimental groups on day 14 (p<0.001). Therefore, better functional recovery was achieved as early as day 14 in animals treated with medication regardless of the kind of medication (
Table 1). After 28 days, SFI values were returned to -7.50±0.00 and -12.52±3.08 in the experimental and the control groups, respectively, meaning that the statistical difference between groups was not significant according to the oneway ANOVA test at this time point (
Table 1).
Histopathological and immunohistochemical findingsFour indices (perineurium development, axonal swelling, leukocyte infiltration, and axon loss) were established to investigate nerve regeneration. Each group received a score for defined indices based on light microscopy evidence in hematoxylin-eosin (
Table 2,
Fig. 1 and
Fig. 2) and toluidine bluestained slides (
Fig. 3).
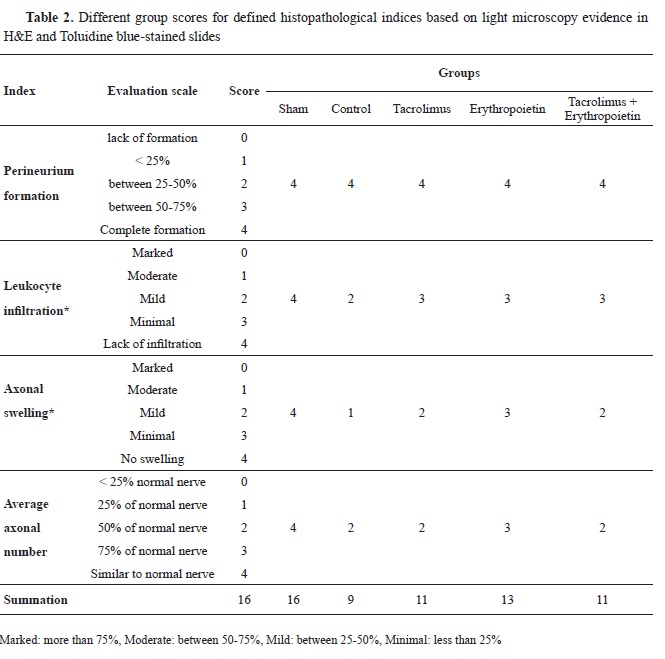
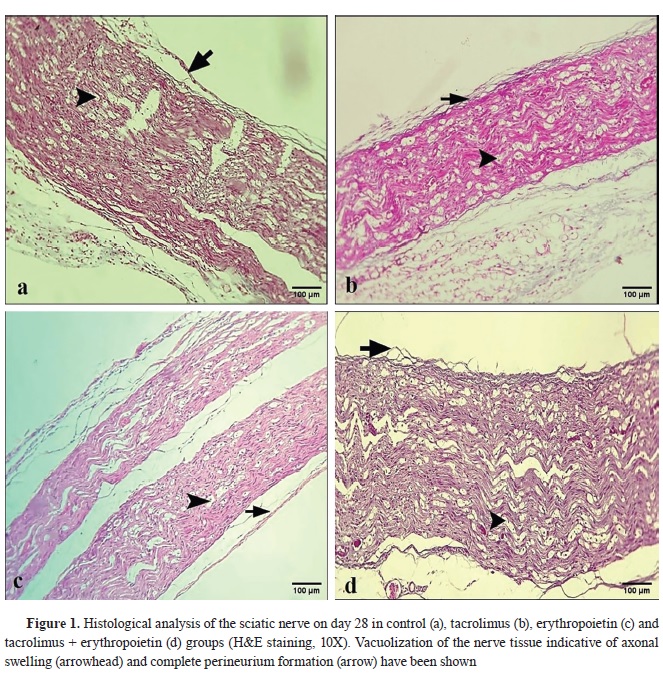
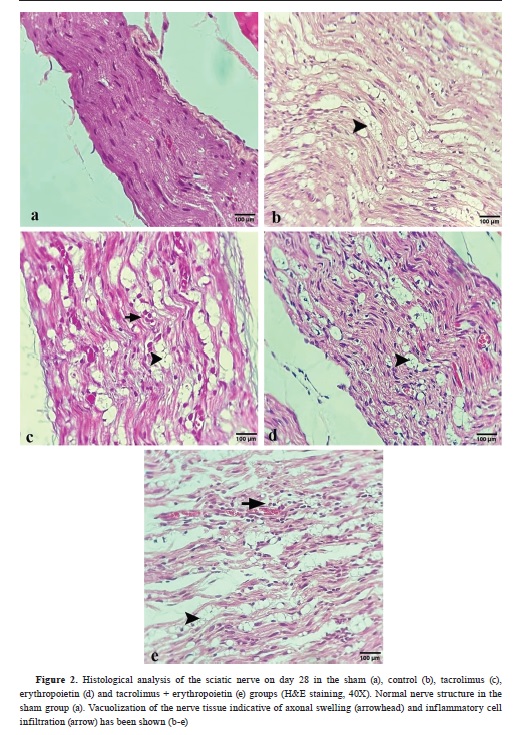
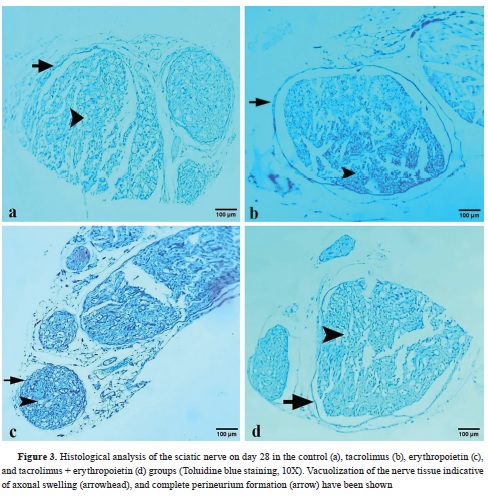
All groups showed complete perineurium formation (
Table 2). There was no statistically significant difference regarding this index among either of the 5 groups based on the Kruskal-Wallis analysis (p=1).
Concerning leukocyte infiltration, no inflammatory cell infiltration was present in the sham group. However, mild and minimal infiltration was observed in the control and experimental groups respectively (
Table 2). Using the Kruskal-Wallis test, the statistical difference among the 5 groups, was significant in this respect (p<0.001). According to the Mann-Whitney U test, inflammatory cell infiltration was significantly different between the sham and other groups (p=0.036). Furthermore, a significant difference was present between the control and experimental groups regarding this index (p=0.008). However, no significant statistical difference was noted between tacrolimus and erythropoietin groups (p=1) and also between tacrolimus + erythropoietin and either of the drugs individually (p=1).
Intergroup evaluation of axonal swelling using Kruskal-Wallis test, showed a significant statistical difference (p<0.001). None of the groups showed significant swelling (score 0, more than 75% axonal diameter). No axonal swelling was present in the sham group, unlike the other groups (
Table 2). The statistical difference between the sham and other groups was significant according to the Mann- Whitney U test (p=0.036). The greatest amount of axonal swelling (more than 75% axonal diameter) was found in the control group, which was significantly higher than the other groups (p<0.05).
The lowest score (minimal, less than 25% axonal diameter) was attributed to the erythropoietin group (
Table 2). Axonal swelling was significantly lower in the erythropoietin group relative to both tacrolimus and tacrolimus + erythropoietin groups (p=0.008). The degree of axonal swelling was equal in the tacrolimus and tacrolimus + erythropoietin groups (mild) and no significant difference was noted between them (p=1) (
Table 2). The average axonal number in the sham group was equivalent to the normal nerve. Tacrolimus and tacrolimus + erythropoietin groups demonstrated half the normal extent for normal nerve in each microscopic field, which was equivalent to the control group. The average axonal number was recovered up to 75% of the normal nerve in the erythropoietin group (
Table 2). Comparing this index via the Kruskal- Wallis test demonstrated significant statistical differences between the groups (p<0.001). According to the Mann-Whitney U test, the sham group axonal count recovery was significantly higher than the other groups (p=0.036). Moreover, there was no significant difference regarding this index between the control and experimental groups (p=1), except for the erythropoietin group which exhibited significantly higher axonal count recovery (p=0.008). In addition, a significantly higher average axonal number was attained in the erythropoietin group compared to both tacrolimus and tacrolimus + erythropoietin groups (p=0.008). The average axonal number wasn’t significantly different between tacrolimus and tacrolimus + erythropoietin groups (p=1).
Table 3, compares GFAP antibody and S-100 expression among different groups. No immunoreactivity to GFAP was found in the sham group (
Fig. 4a). Immunoreactivity to GFAP was marked (+++) in the tacrolimus and moderate (++) in the control, erythropoietin, and tacrolimus + erythropoietin groups (
Fig. 4 b-e). Immunoreactivity to S-100 protein was observed in the regenerated nerves in all groups (
Fig. 5 f-j). S-100 protein expression was as follows: marked cytoplasmic staining (+++) in the tacrolimus, tacrolimus + erythropoietin, and the control groups, and moderate cytoplasmic staining (++) in both the erythropoietin and sham groups.
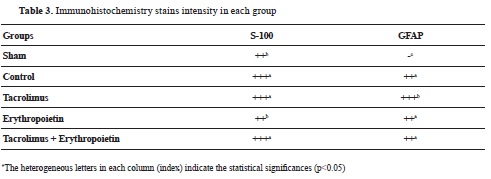
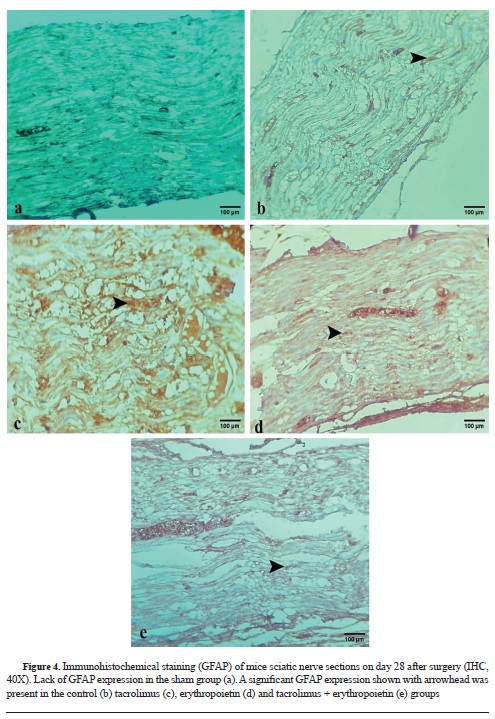
Macroscopic evaluationThere was no evidence of self-mutilation, infection, or inflammatory reaction in either of the groups. Muscle adhesion was marked (+++) in the sham and control groups, moderate (++) in the tacrolimus, and weak (+) in the erythropoietin groups. Muscle adhesion was weak (+) in the tacrolimus + erythropoietin group except for two of the animals in which one demonstrated moderate (++) adhesion along with petechiae on the superficial aponeurosis of the muscle and the other one showed a very small abscess between muscle planes. Tissue derangement was weak (+) in both the sham and control groups, moderate (++) in the tacrolimus group, and weak (+) in the erythropoietin and tacrolimus + erythropoietin groups. Concerning nerve adherence to the surrounding muscles, none of the groups showed marked (+++) adherence requiring sharp dissection with scissors. Adherence of the nerve to the surrounding muscles was negative (-) in the sham, tacrolimus, tacrolimus+erythropoietin, and erythropoietin groups, so the nerve was easily separable from the adjacent tissue. However, weak (+) muscle-to-nerve adherence was noted in the control group, making the dissection of the nerve slightly difficult. Additionally, complete skin and muscle-fascia closure (+) was noted in all of the animals.
DISCUSSION
Peripheral nerve injury remained among the greatest unresolved clinical problems due to high rates of occurrence, slow and imperfect regeneration, long-term morbidity, considerable individual or social costs, and dramatic reduction of patient quality of life (
1, 6). Despite ongoing comprehension of nerve damage pathophysiology, the advent of microsurgical repair, and refinements in the clinical management of nerve injuries, to date, the outcomes of nerve regeneration have still not reached their zenith (
4, 8). Recently, medical therapy has been a promising approach targeting axonal regrowth and functional recovery, especially in crushing nerve injuries, which generally undergo good regeneration (
9, 14, 25). However, no ideal therapeutic agent has been approved yet (
2, 4). This provides a strong impetus to examine new therapeutic agents.
Tacrolimus (FK506) was originally isolated in 1984 (
18, 19). Many reports clarified its critical neuro-regenerative properties besides potent immunosuppressive actions (
16, 17, 30); the earliest one belongs to Gold et al. who reported that tacrolimus increases nerve regeneration in-vivo. Concurrently, Lyon et al. reported that tacrolimus increases in vitro neurite out-growth (
16, 17, 18). Several studies suggested the advantages of both local (
11, 17, 31) and systemic (
17, 18, 19) tacrolimus, however, drawing certain conclusions from them is difficult due to the unique pharmacokinetics in models along with different doses, administration routes, and evaluating methods. Shahraki et al. confirmed the positive effects of systemic tacrolimus (
32). Que et al. declared that tacrolimus reduces scar tissue formation by inducing apoptosis in fibroblasts (
27). Yang et al. reported that tacrolimus induces neuro-regenerative influences in doses lower than 2 mg/kg after repairing transected sciatic nerve (
30). Additionally, the bimodal dose-dependent effect of systemic tacrolimus following sciatic crush and grafting was demonstrated in two separate studies (
33). The fastest regeneration rate was obtained using 5 mg/kg dosage (
33). In the present study, 5 mg/kg systemic tacrolimus decreased leukocyte infiltration and axonal swelling relative to the control group (
Table 2). In contrast to our results, de Mesquita Coutinho et al. gained no histopathologic benefits from the administration of tacrolimus, yet did not discard possible benefits of the drug (
34).
Another multi-purpose nutrient with expanding body of evidence suggesting its neurotrophic, neuroprotective, and neuro-regenerative effects is erythropoietin (
7, 22). Multiple studies addressed its desirable roles not only in the CNS but also in peripheral nerve regeneration (
23, 34, 35). For instance, systemic erythropoietin has been investigated following various degrees of sciatic nerve injury and erythropoietin-receptor positivity enhancement was reported in the nerves that recovered fastest (
7). Furthermore, Sundem et al. claimed that erythropoietin directly protects myelin and promotes myelination in vivo (
23). According to present data, erythropoietin exerted a remarkable axonal count recovery compared to tacrolimus, tacrolimus + erythropoietin, and control groups (
Table 2). Additionally, it significantly decreased axonal swelling compared to both saline and tacrolimus while the reduction of inflammatory cell infiltration was only significant relative to saline.
Hitherto, studies measured the success of peripheral nerve regeneration by morphometric, electrophysiologic, and functional methods (
10, 12, 30). Since proper gait requires a coordinated function of sensory terminals, motor responses, and brain cortex integrity, walking track analysis has been appropriate for evaluating functional recovery (
13, 15, 28). Medinaceli and colleagues (1982) were the first to analyze walking tracks. Further, Bain et al. (1989) reconsidered and ameliorated their proposed equation (
28). The original SFI calculation developed by Medinaceli and Bain was for rats, but Inserra et al. introduced a specialized equation for mice (
28). Several reports focused on the benefits of sole tacrolimus and erythropoietin in functional recovery. For instance, short-term tacrolimus administration (10 days) was sufficient to reduce tibial nerve functional recovery duration by 29% and 15% in the transection and graft models compared to the negative controls, respectively (
36). Moreover, enhanced sciatic functional recovery was reported following 16 weeks of tacrolimus treatment (
32). Furthermore, an initial stimulated functional recovery was pointed out from continuous erythropoietin administration for 4-weeks post-operation and a rapid deterioration was seen following erythropoietin discontinuation (
35). Elfar et al. (
7) reported that sciatic functional recovery was 60% better in mice who received erythropoietin. Additionally, the capacity of single-dose erythropoietin to accelerate functional return subsequent to a moderate sciatic crush was clarified (
21). The present study evaluated the functional effects of erythropoietin (5000 U/ Kg) and tacrolimus (5 mg/kg) administration for 28 days after sciatic crush. Statistical significance was observed concerning functional recovery among the control and all medication groups at the midpoint of the study, however, tacrolimus showed the maximum effect (
Table 1). In line with Lykissas et al. (
35), continuous administration of erythropoietin for 14 days was beneficial although it was not as potent as tacrolimus at this time point. Numerous researchers inspected the expression of various immunohistochemical markers in order to trace nerve regeneration (
27, 32, 37). For instance, Mekaj et al. revealed immunoreactivity to S-100 in the sciatic nerve longitudinal sections treated with topical tacrolimus and hyaluronic acid (
37). Moreover, Shahraki et al. observed extensive immunoreactivity to S-100 in the sciatic nerve cross-sections (
32). According to our results, S-100 and GFAP expression was pronounced in the tacrolimus group. Nevertheless, at least moderate GFAP and S-100 expression was present in all of the groups except the sham highlighting the role of these markers in the healing of injured nerves (
Table 3,
Fig. 4 and
Fig. 5).
To date, given the multi-dimensional and complex nature of nerve injury and regeneration, combining pharmacological therapies has gained researchers’ attention willing to recruit their possible synergism on peripheral nerve regeneration (
18, 31, 38). For instance, Labroo et al. uncovered the potentiating effects of tacrolimus, nerve growth factor (NGF), and glial cell-derived neurotrophic factor on neurite elongation compared to their corresponding individual doses (
31). The synergistic effects of tacrolimus and NGF on sciatic functional recovery have also been clarified (
39). Furthermore, Uzun et al. (
24) compared artesunate, dexamethasone, and tacrolimus effects on the sciatic compressive injury. Contrary to our results suggesting superior tacrolimus capacity in functional recovery, they observed no significant difference among these agents (
24). Moreover, according to Ulger et al. (
38), the SFI values of rats treated with both erythropoietin and umbilical cord-derived mesenchymal stem cells were significantly better than those untreated on day 14. Our results on day 14 were almost in agreement with them because the functional recovery of medication groups was significantly better than the control group, however, the best SFI values were recorded for tacrolimus and not tacrolimus + erythropoietin combination (
Table 1). In addition, Lee et al. (
40) implied that dexamethasone associated with erythropoietin significantly improved functional return as early as day 3, although either of the agentsa and their combination were capable of improving function compared to the control group on day 28. Based on our results on day 28, although functional recovery was mildly improved in groups treated with either tacrolimus, erythropoietin, or both versus the control group this was not statistically significant (
Table 1). In another study decellularized allografts treated with tacrolimus and NGF was implanted in sciatic nerve gaps. An improved allograft uniformity in groups that received treated allografts but a swollen tangled mass in the pure allograft group was mentioned and this was interpreted as connective tissue fibrosis preventing effective functional recovery (
39). In line with their results, we observed muscle-to-nerve adherence only in the control group. If this is taken into consideration as fibrosis, tacrolimus, and erythropoietin may be capable of decreasing connective tissue formation. Additionally, based on gross observations, tissue derangement was lower in mice that received erythropoietin relative to tacrolimus.
According to our literature review, a single study investigated the combination of tacrolimus and erythropoietin on the spinal cord injury rather than peripheral nerve (
34). They failed to show any synergism between tacrolimus and erythropoietin. Since, as opposed to our results indicating a favorable functional return in the tacrolimus group, they yielded faster and better functional recovery in the erythropoietin group (
34).
To the best of our knowledge, the effects of simultaneous administration of tacrolimus and erythropoietin on peripheral nerves especially sciatic nerve injury have not been studied yet. In the present study, we assessed their individual and possible synergistic influences on sciatic crush. We confirmed that coadministration of tacrolimus and erythropoietin led to statistically significant improved functional recovery in comparison to the control group. From the histopathologic point of view, tacrolimus + erythropoietin reduced inflammatory cell infiltration, but this was only significant relative to the control group. Erythropoietin decreased axonal swelling and axonal loss score, however, there was no synergistic effect between tacrolimus and erythropoietin in this context (
Table 2). Furthermore, tacrolimus induced better GFAP and S-100 expression compared to erythropoietin and the combination of these two performed better relative to erythropoietin alone (
Table 3,
Fig. 4 and
Fig. 5). Therefore, functionally tacrolimus performed better whereas histopathologically erythropoietin was preferable.
CONCLUSION
Altogether, this study demonstrated some ameliorative effects of systemically administered tacrolimus and erythropoietin on sciatic nerve regeneration that may encourage their application in selected conditions. Unfortunately, enough data was not gained regarding the histopathologic, immunologic, or functional synergism of both drugs at least by the dose, route, and duration used. Whether actual synergism does exist between erythropoietin and tacrolimus requires further evaluation. Given almost the maximum effect of the drugs on day 14 and SFI recovery by more than 80% after 28 days even in the control group, it may be inferred that the study time point was too long for assessing sciatic nerve crush injury. In the author’s opinion, better histopathological and immunohistochemical results would be achieved if they have been evaluated earlier in the course of the study. Further detailed experimental studies are warranted to answer the unresolved questions about tacrolimus and erythropoietin in combination.
CONFLICT OF INTEREST
The authors declare that they have no potential conflict of interest with respect to the authorship and/or publication of this article.
ACKNOWLEDGMENTS
This research was supported by Faculty of Specialized Veterinary Sciences, Science and Research Branch, Islamic Azad University. The authors would like to acknowledge Dr. Pezhman Mortazavi, Dr. Nikta Mansouri, Dr. Bita Vazir, Dr. Niusha Pahlevaninezhad, and Dr Mahya Jazini for their kind cooperation and contribution to this study.
AUTHORS’ CONTRIBUTIONS
KM participated in study conceptualization and design, data acquisition, provision of financial support, provision of study material, medication preparation and wrote the manuscript. HF participated in study conceptualization and design, provision of study material, instrumentation, financial support, and performed the surgery. AJ was involved in histopathological and immunohistochemical analysis and the provision of laboratory samples. HA performed the statistical analysis and interpreted the results. All authors reviewed the results and approved the final version to be published.